Igbt Gate Driver
When the Insulated Gate Bipolar Transistor (IGBT) was invented by Professor Jayant Baliga in 1980, it was seen as an ideal combination of the low on-state saturation voltage of a bipolar transistor and ease of gate drive of a MOSFET. IGBTs now find their natural home in applications at high power using devices with effective gate capacitances measured in hundreds of nanofarads. Although this capacitance has simply to be charged and discharged to turn the IGBT on and off, the circulating current to do so causes significant power dissipation in voltage drops in the gate driver circuit and within the IGBT. When IGBTs are paralleled, the gate drive power required is higher still.
At high power, inverters or converters typically use ‘bridge’ configurations to generate line-frequency AC or to provide bi-directional PWM drive to motors, transformers or other loads. Bridge circuits include IGBTs whose emitters are switching nodes at high voltage and high frequency so the gate drive PWM signal and associated drive power rails, which use the emitter as a reference, have to be ‘floating’ with respect to system ground, so called ‘high side’ drives. Additional requirements are that the drive circuit should be immune to the high ‘dV/dt’ of the switch node and have a very low coupling capacitance. In many instances, the bridge circuit requires safety agency-rated isolation from the control circuitry and therefore the barrier must be robust and show no significant degradation over the design life time due to partial discharge effects.
Technical Article Gate Drivers for SiC-MOSFET/IGBT Power Modules and Their Advantages May 17, 2019 by Hirotoshi Aoki This article highlights Tamura Corporation Gate Drivers module which is the 2DMB series for SiCMOSFET/IGBT power modules that contains DC/DC converters. NXP offers an advanced gate driver for high-voltage power IGBTs which integrates the high-voltage isolator. The MC33GD3100 device offers current and temperature-sense features, including integrated current and temperature-monitoring outputs, that work directly with the new high-current-density IGBT modules. Power Integrations’ SCALE™-2 IGBT Gate Drivers use an ASIC chip set specifically-designed to reduce count, save space and increase product reliability and functionality. The TD350E device is an advanced gate driver for IGBTs and power MOSFETs. Control and protection functions are included and allow the design of high reliability systems. The innovative active Miller clamp function eliminates the need for negative gate drive in most applications and allows the use of a simple bootstrap supply for the high side.
We will now consider how DC-DC converters can provide optimum power rails for these ‘floating’ drive circuits using a typical IGBT from Infineon as an example, type FZ400R12KE4.
On and off drive voltages
An initial consideration is to set the on and off-state gate voltages. While part FZ400R12KE4 has a minimum turn-on threshold of 5.2 Vat 25 Celsius, in practice to ensure full saturation and rated collector current of 400 A, at least 10 V must be applied. The part has a maximum gate voltage of ± 20 V so +15 V is a good value with some margin. Higher values produce unnecessary dissipation in the gate drive circuit.
For the off-state, 0 V on the gate can be adequate. However, a negative voltage typically between -5 and -10 V enables rapid switching controlled by a gate resistor. A consideration also is that any emitter inductance between the IGBT and the driver reference, (point x in Figure 1), causes an opposing gate-emitter voltage when the IGBT is turning off. While the inductance may be small, just 5 nH would produce 5 V at a di/dt of 1000 A/µs which is not unusual. 5 nH is just a few millimetres of wired connection (the FZ400R12KE4 has a stray package inductance of 16 nH). An appropriate negative drive ensures that the gate-emitter off-voltage is always zero or less.
A negative gate drive also helps to overcome the effect of collector-gate ‘Miller’ capacitance on device turn-off which works to inject current into the gate drive circuit. When an IGBT is driven off, the collector-gate voltage rises and current flows through the Miller capacitance of value Cm. dVce/dt into the gate emitter capacitance Cge and through the gate resistor to the driver circuit, see Figure 2. The resulting voltage Vge on the gate can be sufficient to turn the IGBT on again with possible shoot-through and damage. Driving the gate to a negative voltage mitigates this effect.
A DC-DC converter with +15/-9V outputs conveniently provides the optimum voltages for the gate driver.
Gate power requirements
The gate of an IGBT must be charged and discharged through Rg in each switching cycle. If the IGBT data sheet provides a gate charge curve then the relationship is:
P = Qg. F .Vs
Where P is gate drive power, Qg is data sheet charge for a chosen gate voltage swing, positive to negative, of value Vs.
If the data sheet does not provide a charge curve but just a Qg value at specific gate voltages, the value of Qg at other gate voltage swings can be approximated by multiplying by the ratio of the actual versus data sheet voltage swings. For example the FZ400R12KE4 has a Qg value of 3.7 µC with ±15 V gate voltage swing (30 V total). For a swing of +15/-9 V (24 V total) gate charge approximates to:
Qg = 3.7e-6 . 24/30 ≈ 3 µC
At 10 kHz this requires gate drive power of:
Pg = 3e-6 .10e3 . 24 ≈ 0.72 W
With derating and allowing for other incidental losses, a 2 W DC-DC converter would be suitable.
Average current requirements
In our example, with 24 V total gate voltage swing, the charge and discharge energy must be the same in each cycle, so the average charge and discharge current must be the same, at 30 mA given by Pg/Vs.
Peak current requirements
The peak current Ipk, required to charge and discharge the gate is a function of Vs, gate resistance of the IGBT Rint and external resistance Rg.
Ipk = V s/(Rint + Rg)
The FZ400R12KE4 has Rint = 1.9 ohms so with a typical external resistor of 2 ohms and a swing of 24 V, a peak current of over 6 A results. This peak current must be supplied by ‘bulk’ capacitors on the driver supply rails as the DC-DC converter is unlikely to have sufficient value of output capacitors to supply this current without significant ‘droop’. Of course the gate driver itself must be rated for these peak current values as must the gate resistors.
Bulk capacitance values
For our example, total gate drive energy E per cycle is given by:
E = Qg. V s = 72 µJ
The bulk capacitors on the +15 and -9 V rails supply this energy in proportion to their voltages so the +15 V rail supplies 45 µJ. If we assume that the bulk capacitor on the +15 V rail should not drop more than say 0.5 V each cycle then we can calculate minimum capacitance C by equating the energy supplied with the difference between the capacitor energies at its start and finish voltages, that is;
45 µJ = ½ C (Vinit2 – Vfinal2)
C = (45e -6 . 2)/(152 – 14.52) ≈ 6.1 µF
Although the -9 V rail supplies about a third of the energy, it requires the same capacitor value for 0.5 V drop as this is a larger percentage of the initial value.
In practice the voltage drop may be affected more by the ESR and ESL of the capacitor. For example an ESR of 0.1 ohm would drop more than 0.5 V peak at our peak current of 6 A. High performance capacitors should therefore be chosen, often necessitating large can sizes to achieve the low ESR, giving many times the minimum capacitance value.
DC-DC regulation
The absolute values of gate drive voltages are not very critical as long as they are above the minimum, comfortably below breakdown levels and dissipation is acceptable. The DC-DC converters supplying the drive power therefore may be unregulated types if the input to the DC-DCs is nominally constant. Unlike most applications for DC-DCs however, the load is quite constant when the IGBT is switching at any duty cycle. Alternatively the load is close to zero when the IGBT is not switching. Simple DC-DCs often need a minimum load otherwise their output voltages can dramatically increase, possibly up to the gate breakdown level. This high voltage is stored on the positive bulk capacitor so that when the IGBT starts to switch, it could see a gate overvoltage until the level drops under normal load. A DC-DC should be chosen therefore that has clamped output voltages or zero minimum load requirements.
DC-DC converter start-up and shutdown
IGBTs should not be actively driven by PWM signals until the drive circuit voltage rails are at correct values. However, as gate drive DC-DCs are powered up or down, a transient condition might exist where IGBTs could be driven on, even with the PWM signal inactive, leading to shoot-through and damage. The DC-DC should therefore be well behaved with short and monotonic rise and fall times. A primary referenced on-off control can enable sequencing of power-up of the DC-DCs in a bridge reducing the risk of shoot-through.
DC-DC converter coupling capacitance
DC-DCs for ‘high side’ IGBT drives see the switched ‘DC-link’ voltage across their barrier. This voltage can be kilovolts with very fast switching edges from 10 kV/µs upwards. Latest GaN devices may switch at 100 kV/µs or more. This high ‘dV/dt’ causes displacement current through the capacitance of the DC-DC isolation barrier of value:
I = C. dV/dt
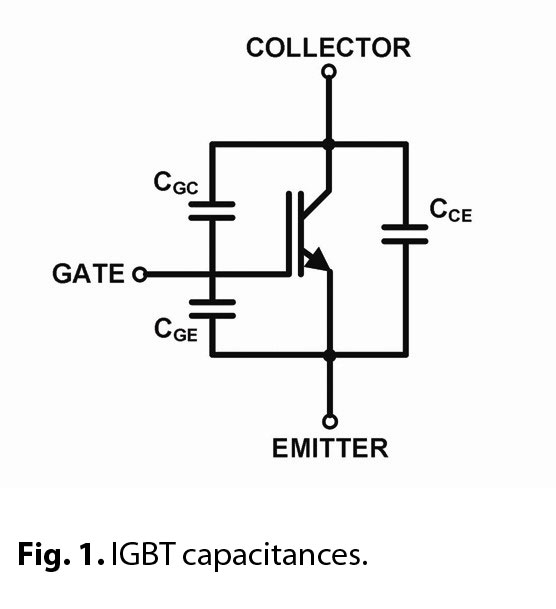
So for just 20 pF and 10 kV/µs, 200 mA is induced. This current finds an indeterminate return route through the controller circuitry back to the bridge causing voltage spikes across connection resistances and inductances potentially disrupting operation of the controller and the DC-DC converter itself. Low coupling capacitance is therefore desirable, ideally less than 15 pF.
Converter isolation
When the IGBT driver is powered by an isolated DC-DC converter, the barrier in the converter will be expected to withstand the switched voltage applied to the IGBTs which may be kilovolts at tens of kHz. Because the voltage is switched, the barrier will degrade over time faster than with just DC by electrochemical and partial discharge effects in the barrier material. The DC-DC converter must therefore have robust insulation and generous creepage and clearance distances. If the converter barrier also forms part of a safety isolation system, the relevant agency regulations apply for the level of isolation required (basic, supplementary, reinforced), operating voltage, pollution degree, overvoltage category and altitude. EN 62477-1:2012 is an appropriate standard to apply for ‘Power Electronic Converter Systems’ where IGBTs would commonly be used.
Temperature ratings
It is advisable to place the IGBT driver and its DC-DC converter as close as possible to the IGBT to minimise noise pick up and volt drops. This places the components in a potentially high temperature environment where reliability and lifetime reduces. DC-DC converters should be chosen with appropriate ratings and without internal components that suffer significantly with temperature such as electrolytic capacitors and opto-couplers. The rated maximum operating temperature for a low power DC-DC converter will normally be specified at maximum load and no forced airflow. If the average load is lower than maximum or there is guaranteed airflow, the converter manufacturer may be able to provide data showing that critical temperatures are not exceeded at higher temperatures. This will not always be the case as the simplest of converters can have much reduced efficiency at lighter loads negating any load derating benefit. If the converter is providing a safety barrier, the user should also look for any conditions of use in the data sheet imposed by the safety agency. This would typically be a maximum operating temperature less than the manufacturer’s maximum for non-safety barrier applications. Consider any thermal shock conditions; a DC-DC converter may work at constant extremes of temperature happily but may fail or degrade if subjected to very high thermal shock.
Operating temperature affects any converter’s failure rate. Data sheet MTTF values will typically be quoted at 25 or 40 Celsius and should be extrapolated for actual operating temperatures. The converter manufacturer should be able to do this easily. Often MIL-HBK 217 is used for reliability prediction as a most pessimistic standard but other standards are sometimes used giving dramatically different answers so comparison of data sheet values should be approached with care.
Alternatives to isolated DC-DC converters
It is not always necessary to use isolated DC-DC converters to provide drive power to IGBTs. If isolation is not required then a cheap and simple charge pump can suffice as shown in Figure 3. C1 is charged through D1 and R1 when Q2 is on. When Q2 is off and Q1 switches on, D1 is reverse biased and C1 now provides a voltage referenced to the upper IGBT emitter to power its driver.
Disadvantages of this approach are:
- No drive voltage available if IGBTs are not switching.
- No isolation to meet safety requirements.
- Only suitable for low power.
- Generation of positive and negative gate drive voltages is more complex.
- Not suitable for ‘normally on’ devices.
- Diode D1 must be high voltage and have very low reverse recovery current.
- R1 is necessary to limit high peak current into C1 but affects C1 voltage as duty cycle changes.
- No continuous power for control and monitoring (e.g. desaturation detection).
Another alternative to an isolated DC-DC converter is to drive the gate of the IGBT directly from the PWM signal through a transformer as shown in Figure 4.
Disadvantages of this approach are:
- Drive voltages depend on duty cycle without C1, C2, D1 and D2 to provide ‘DC restoration’ and constant positive/negative drive voltages.
- C1, C2 have to ‘settle’ to DC offsets on start-up and with changes in duty cycle. See Figure 5.
- The transformer may have to deliver significant power (watts) so may be large and unsuitable for high frequencies (IGBT gate power requirement increases with frequency).
- A large transformer has high coupling capacitance giving high circulating currents from dV/dt across the transformer.
- Transformers designed for low coupling capacitance have high leakage inductance limiting PWM slew-rate.
- The transformer requires special construction to meet any safety agency standards for isolation.
- The transformer primary has to be driven by a high speed buffer for high power which is complex and costly for good bandwidth.
- Not suitable for ‘normally on’ devices.
- No continuous power for control and monitoring (e.g. desaturation detection).
- Transformer must ‘reset’ between pulses – must consider pulse width limits and reset voltage limits.
Figure 5 shows a simulation of the typical effect on the gate drive voltage of C1 and C2 resonantly settling to their final average DC offsets after switch on to 80% duty cycle. On-state drive voltages are seriously distorted risking IGBTs being insufficiently saturated for the duration. Sudden changes in duty cycle produce the same effect during normal operation.
Conclusion
DC-DC converters providing gate drive power for IGBTs need specific performance characteristics but provide significant advantages over alternative techniques in obtaining optimum IGBT efficiency and security under all start-up, transient and continuous operating conditions.
Related products
Related articles
Abstract
An IGBT/power MOSFET is a voltage-controlled device that is used as a switching element in power supply circuits and motor drives, amongst other systems. The gate is the electrically isolated control terminal for each device. The other terminals of a MOSFET are source and drain, and for an IGBT they are called collector and emitter. To operate a MOSFET/IGBT, typically a voltage has to be applied to the gate that is relative to the source/emitter of the device. Dedicated drivers are used to apply voltage and provide drive current to the gate of the power device. This article discusses what these gate drivers are, why they are required, and how their fundamental parameters, such as timing, drive strength, and isolation, are defined.
Need for a Gate Driver
The structure of an IGBT/power MOSFET is such that the gate forms a nonlinear capacitor. Charging the gate capacitor turns the power device on and allows current flow between its drain and source terminals, while discharging it turns the device off and a large voltage may then be blocked across the drain and source terminals. The minimum voltage when the gate capacitor is charged and the device can just about conduct is the threshold voltage (VTH). For operating an IGBT/power MOSFET as a switch, a voltage sufficiently larger than VTH should be applied between the gate and source/emitter terminals.
Consider a digital logic system with a microcontroller that can output a PWM signal of 0 V to 5 V on one of its I/O pins. This PWM would not be enough to fully turn on a power device used in power systems, as its overdrive voltage generally exceeds the standard CMOS/TTL logic voltage. Thus, an interface is needed between the logic/control circuitry and the high power device. This can be implemented by driving a logic level n-channel MOSFET, which, in turn, can drive a power MOSFET as seen in Figure 1a.
As in Figure 1a, when IO1 sends out a low signal, VGSQ1 < VTHQ1 and, thus, MOSFET Q1 remains off. As a result, a positive voltage is applied at the gate of power MOSFET Q2. The gate capacitor of Q2 (CGQ2) charges through pull-up resistor R1 and the gate voltage is pulled to the rail voltage of VDD. Given VDD > VTHQ2, Q2 turns on and can conduct. When IO1 outputs high, Q1 turns on and CGQ2 discharges through Q1. VDSQ1 ~ 0 V such that VGSQ2 < VTHQ2 and, hence, Q2 turns off. One issue with this setup is of power dissipation in R1 during on state of Q1. To overcome this, pMOSFET Q3 can be used as a pull up to operate in a complementary fashion with Q1, as seen in Figure 1b. PMOS has a low on state resistance and with its very high resistance in the off state, power dissipation in the drive circuit is greatly reduced. To control edge rates during gate transition, a small resistor is externally added between drain of Q1 and gate of Q2. Another advantage of using a MOSFET is the ease of fabricating it on a die as opposed to fabricating a resistor. This distinct interface to drive the gate of a power switch can be created in the form of a monolithic IC, which accepts a logic-level voltage and generates a higher power output. This gate driver IC will almost always have additional internal circuits for greater functionality, but it primarily works as a power amplifier and a level shifter.
Key Parameters of a Gate Driver
Drive Strength:
The issue of providing appropriate gate voltage is addressed by using a gate driver that does the job of a level shifter. The gate capacitor though, cannot change its voltage instantaneously. Thus, a power FET or IGBT has a non-zero, finite switching interval. During switching, the device may be in high current and high voltage state, which results in power dissipation in the form of heat. Thus, transition from one state to another needs to be fast so as to minimize switching time. To achieve this, a high transient current is needed to charge and discharge the gate capacitor quickly.
A driver that can source/sink higher gate current for a longer time span produces lower switching time and, thus, lower switching power loss within the transistor it drives.
The source and sink current rating for the I/O pins of a microcontroller is typically up to tens of milliamps, whereas gate drivers can provide much higher current. In Figure 2, a long switching interval is observed when a power MOSFET is driven by a microcontroller I/O pin at its maximum rated source current. As seen in Figure 3, transition time reduces significantly with an ADuM4121 isolated gate driver, which provides much higher drive current than a microcontroller I/O pin, drives the same power MOSFET. In many cases, driving a larger power MOSFET/IGBT directly with a microcontroller might overheat and damage the control due to a possible current overdraw in the digital circuit. A gate driver with higher drive capability enables fast switching with rise and fall times of a few nanoseconds. This reduces the switching power loss and leads to a more efficient system. Hence, drive current is usually considered to be an important metric in selection of gate drivers.
Corresponding to the drive current rating is the drain-source on-resistance (RDS(ON)) of a gate driver. While ideally the RDS(ON) value should be zero for a MOSFET when fully on, it is generally in the range of a few ohms due to its physical structure. This takes into account the total series resistance in the current flow path from drain to source.
RDS(ON) is the true basis for maximum drive strength rating of a gate driver, as it limits the gate current that can be provided by the driver. RDS(ON) of the internal switches determines sink and source current, but external series resistors are used to reduce drive current and, thus, affect edge rates. As seen in Figure 4, high-side on-resistance and the external series resistor REXT form the gate resistor in the charging path, and low-side on-resistance with REXT forms the gate resistor in the discharging path.
RDS(ON) also directly affects power dissipation internal to the driver. For a specific drive current, the lower value of RDS(ON) allows higher REXT to be used. As power dissipation is distributed between REXT and RDS(ON),the higher value of REXT implies more power is dissipated external to the driver. Hence, to improve system efficiency and to relax any thermal regulation requirement within the driver, the lower value of RDS(ON) is preferred for the given die area and size of the IC.
Timing:
Gate driver timing parameters are essential to evaluate its performance. A common timing specification for all gate drivers including ADuM4120—shown in Figure 5—is the propagation delay (tD) of the driver, which is defined as the time it takes an input edge to propagate to the output. As in Figure 5, rising propagation delay (tDLH) may be defined as the time between the input edge rising above the input high threshold (VIH) to the output rising above 10% of its final value. Similarly, falling propagation delay (tDHL) can be stated as the time from the input edge falling below input low threshold VIL to the time output falls below 90% of its high level. The propagation delay for output transition can be different for a rising edge and a falling edge.
Figure 5 also shows the rise and fall times of the signal. These edge rates are affected by the drive current that a part can deliver, but they are also dependent on the load being driven and are not accounted for in propagation delay calculation. Another timing parameter is pulse width distortion, which is the difference between rising and falling propagation delay on the same part. Thus, pulse width distortion (PWD) = tDLH – tDHL .
Igbt Gate Driver Module
Due to mismatch between transistors within different parts, the propagation delay on two parts will never exactly be same. This results in propagation delay skew (tSKEW), which is defined as the time difference between output transitions on two different parts when reacting to the same input in the same operating conditions. As seen in Figure 5, propagation delay skew is defined as part-to-part. For parts that have more than one output channel, this specification is stated in the same way, but is noted as channel-to-channel skew. Propagation delay skew cannot usually be accounted for in the control circuit.
Figure 6 shows a typical setup of ADuM4121 gate drivers used with power MOSFETs in a half-bridge configuration for power supplies and motor drive applications. In such a setup, if both Q1 and Q2 are on at the same time, there is a chance of shoot-through due to the shorting of supply and ground terminals. This can permanently damage the switches and even the drive circuit. To avoid shoot-through, a dead-time must be inserted in the system so that the chance of both switches being on at once is greatly reduced. During the dead-time interval, gate signal to both switches is low and, thus, the switches are ideally in off state. If propagation delay skew is lower, the dead-time required is lower and control becomes more predictable. Having lower skew and lower dead-time results in smoother and more efficient system operation.
Timing characteristics are important, as they affect the speed of operation of the power switch. Understanding these parameters leads to an easier and more accurate control circuit design.
Isolation:
It is the electrical separation between various functional circuits in a system such that there is no direct conduction path available between them. This allows individual circuits to possess different ground potentials. Signal and/or power can still pass between isolated circuits using inductive, capacitive, or optical methods. For a system with gate drivers, isolation may be necessary for functional purposes and it might also be a safety requirement. In Figure 6, we could have VBUS of hundreds of volts with tens of amperes of current passing through Q1 or Q2 at a given time. In case of any fault in this system, if the damage is limited to electronic components, then safety isolation may not be necessary, but galvanic isolation is a requirement between the high power side and low voltage control circuit if there is any human involvement on the control side. It provides protection against any fault on the high voltage side as the isolation barrier blocks electrical power from reaching the user in spite of component damage or failure.
Isolation is mandated by regulatory and safety certification agencies in order to prevent shock hazard. It also protects low voltage electronics from any damage due to faults on the high power side. There are various ways to describe safety isolation, but at a fundamental level, they all relate to the voltage at which the isolation barrier breaks down. This voltage rating is generally given across lifetime of the driver, as well as for voltage transients of a specific duration and profile. These voltage levels also correspond to the physical dimensions of the driver IC and the minimum distance between pins across the isolation barrier.
Apart from safety reasons, isolation may also be essential for correct system operation. Figure 6 shows a half-bridge topology commonly used in motor drive circuits where only one switch is on at a given time. At the high power side, low-side transistor Q2 has its source connected to ground. The gate-source voltage of Q2 (VGSQ2) is thus directly referenced to ground and the design of the drive circuit is relatively straightforward. This is not the case with high-side transistor Q1, as its source is the switching node, which is pulled to either the bus voltage or ground depending on which switch is on. To turn on Q1, a positive gate to source voltage (VGSQ1) that exceeds its threshold voltage should be applied. Thus, gate voltage of Q1 would be higher than VBUS when it is in on state as the source connects to VBUS. If the drive circuit has no isolation for ground reference, a voltage larger than VBUS will be required to drive Q1. This is a cumbersome solution that is not practical for an efficient system. Thus, control signals that are level shifted and referenced to the source of the high-side transistor are required. This is known as functional isolation and it can be implemented using an isolated gate driver, such as ADuM4223.
Noise Immunity:
Gate drivers are used in industrial environments that inherently have a lot of noise sources. Noise can corrupt data and make a system unreliable, leading to degraded performance. Thus, gate drivers are required to have good immunity to noise to ensure data integrity. Noise immunity pertains to how well the driver rejects electromagnetic interference (EMI) or RF noise and common-mode transients.
EMI is any electrical noise or magnetic interference that disrupts the expected operation of the electronic device. EMI, which affects gate drivers, is a result of high frequency switching circuits and is mainly created due to the magnetic field from large industrial motors. EMI may be radiated or conducted and can couple into other nearby circuits. Hence, immunity to EMI or RF immunity is a metric that refers to the ability of a gate driver to reject electromagnetic interference and maintain robust operation without errors. Having high EMI immunity allows drivers to be used in close proximity to large motors without introducing any faults in data transmission.
Igbt Gate Driver Circuit Diagram
As seen in Figure 6, the isolation barrier is expected to provide high voltage isolation across grounds at different potentials. But high frequency switching results in short edges for voltage transitions on the secondary side. These fast transients are coupled from one side to the other due to parasitic capacitance between the isolation boundary, which can lead to data corruption. This can be in the form of introducing jitter in the gate drive signal or inverting the signal altogether, leading to poor efficiency or even shoot-through in some cases. Thus, a defining metric for gate drivers is common-mode transient immunity (CMTI), which quantitatively describes the ability of an isolated gate driver to reject large common-mode transients between its input and output. The immunity of a driver needs to be high if the slew rates in the system are high. Thus, CMTI numbers are particularly significant when operating at high frequencies and large bus voltages.
Igbt Gate Driver Circuit
Conclusion
Igbt Gate Driver Ic
This article is intended to provide an introduction to gate drivers and, thus, the parameters discussed so far do not form an exhaustive list with regard to isolated gate driver specifications. There are other driver metrics such as supply voltage, allowable temperature, pinout, etc. that are a common consideration as with every electronic part. Some drivers, such as ADuM4135 and ADuM4136, also incorporate protection features or advanced sensing or control mechanisms. The variety of isolated gate drivers available in the market make it imperative for a system designer to understand all these specifications and features to make an informed decision about using appropriate drivers in relevant applications.